近视眼的巩膜重塑及其操作:巩膜加固和近视控制的最新进展综述
前言
巩膜是一种特殊的结缔组织,占眼球外层表面积的大部分。因此,眼睛大小的任何变化都必须由巩膜的组织体积和(或)表面积的变化来推动。由于眼轴大小是屈光不正的主要决定因素[1],眼睛和屈光发育领域的研究人员长期以来一直对巩膜很感兴趣。特别是,人们一直致力于了解巩膜的结构、结构如何变化能够促进眼睛大小的变化、什么因素影响或控制这些变化,以及这些变化是主动的还是被动的。最近,人们对调节和促进巩膜结构变化的生物分子和遗传介质特别感兴趣。
也许人们对巩膜产生兴趣的主要原因是其在近视发展中的作用,更具体的原因是(I) 近视已经成为世界上一个主要的社会经济问题,也是主要的公共卫生问题[2];(II) 高度近视与眼镜的大小有关,在其中眼部脆弱结构上的压力会导致不可逆转的损伤,并最终导致失明[3]。因此,努力了解巩膜参与眼球过度生长过程的最终目标,是应用这些知识来干预眼部生长机制,最终防止视力丧失并减少近视管理的社会经济负担。
从最早的人类近视研究开始,人们就知道随着近视的发展巩膜会明显变薄,尤其是在眼球的后极部[4]。因此,大多数干预近视发展的试验和临床方法都是为了减缓或逆转巩膜变薄[5],或者加强因变薄而变脆弱的巩膜[6]。多年来,通过开发近视的动物模型[7],该领域取得了重大进展。通过动物模型可以深入调查近视发展的生物学机制,而这通常无法通过死后的人体组织实现。特别是,动物模型使我们对近视发展和进展过程中巩膜重塑的机制有了进一步的了解,并且促进了对巩膜重塑可能被减缓、阻止或在某些情况下被逆转的方法学探索[8]。因此,本综述将考虑我们对近视眼巩膜重塑过程的最新认识,以及控制重塑和加强巩膜的最新进展。
巩膜形态和基础生物学
巩膜是一种致密的、不规则的结缔组织,由高度组织化和紧密调节的胶原细胞外基质组成。在哺乳动物中,它约占眼球表面积的85%,前方是角膜,后方是进入眼球的视神经[9]。哺乳动物的巩膜是纤维化的细胞外基质,而在禽类和其他脊椎动物中,可以看到更复杂的巩膜结构。巩膜结构通常由较薄的纤维层构成,这与哺乳动物的纤维层相似,有较厚的软骨层,并提供结构中的大部分抗拉强度[5]。这篇综述将主要集中在最能反映人类巩膜结构的哺乳动物的纤维结构上,但是由于禽类薄纤维层与哺乳动物巩膜结构的相似性,这些研究也提供了许多有用的信息,因此这篇综述也会参考禽类近视模型的研究。
巩膜细胞外基质主要由平行但相互交织的胶原纤维束组成[10],虽然在整个巩膜结构的大部分区域,其方向是随机的,但在局部区域却表现出方向的选择性,这可能与局部的拉伸要求(如眼外肌的附着区域)有关[9]。通常发现胶原束在巩膜的内部和外部的厚度上有所不同[10]。最近的研究表明,这些纤维束的巩膜内侧显现不太明显的卷曲,而在巩膜外侧显示出正弦形的 “波浪状”或卷曲[11]。此外,这些纤维束主要由横截面直径在28至280纳米之间的胶原纤维组成,与巩膜外侧相比,巩膜内侧的纤维束的平均直径通常会减少[12]。
在分子水平上,巩膜胶原蛋白纤维主要由I型胶原蛋白分子组成,其中夹杂着至少11种其他类型的胶原蛋白,它们在维持结构、调节原纤维大小和介导原纤维间的相互作用方面发挥着不同的作用[13]。尽管已知胶原蛋白占哺乳动物巩膜干重的80%以上[14],但还有其他一些重要的蛋白和多糖分子存在于巩膜基质中,对巩膜的平衡非常重要。巩膜基质中含有一系列大大小小的蛋白聚糖分子,它们也有助于纤维结构,介导纤维之间的相互作用并控制巩膜基质的水化[15]。虽然关于巩膜结构的文章较少,但已知胶原纤维束松散地包含在小胶原纤维和弹性纤维的微纤维鞘内[10],纤维束间的空间包含较短的弹性纤维[11],而且可能是充满液体的空隙。
束间和纤维间空间含有许多对巩膜细胞外基质结构和功能非常重要的酶和调节剂,其中包括一系列控制基质重塑的酶,如胶原降解酶、基质金属蛋白酶、组织金属蛋白酶抑制剂家族的调节剂[16],以及一系列控制细胞外基质成分表达的细胞因子和其他信号分子,包括转化生长因子β(TGF-β)家族的成员[17]。成纤维细胞是巩膜细胞外基质的常驻细胞,负责巩膜基质结构的产生、组织和调节。然而,巩膜成纤维细胞群中有很大一部分是完全分化的肌成纤维细胞[18],它们通过富含α-平滑肌肌动蛋白(α-SMA)的细胞骨架,通过整合素粘附分子[19]与细胞外基质进行桥接,这有可能对巩膜内的生物力学调节作出贡献。
鉴于巩膜对近视眼过度生长的重要性显而易见,因此对上述每个要素进行了广泛的研究也就不足为奇了,特别是它们各自在近视眼中如何变化。这些研究是通过使用近视动物模型直接进行,也有通过对人类近视者死后巩膜组织的观察间接进行。
近视眼巩膜的形态和生物化学改变
高度近视眼巩膜的特征是明显变薄,有时厚度接近正视眼巩膜的一半[4]。巩膜过度变薄与人类高度近视中的后巩膜葡萄肿有关,也与这些人的近视程度有关[20]。然而,近视动物的相关研究提示,后部巩膜的普遍变薄可能是所有近视发展的一个特征[12]。在超微结构层面上,这种巩膜的总体形态变化与基质中直径较小的胶原纤维占多数有关,导致巩膜内侧和外侧的平均纤维直径梯度减少。在近视眼中,整个巩膜厚度的胶原纤维束更少,这些纤维束也更薄[12]。因此,近视眼巩膜组织特定区域的干重低于同种眼的类似区域,这并不令人惊讶。然而,近视的动物模型也表明,这种巩膜变薄和组织损失不仅仅是一种局部现象,因为巩膜的整体干重也会减少,这些结果表明随着近视的发展,组织会主动损失,而不仅仅是眼球增大时组织简单的重新分布[8]。
尽管对高度近视人眼的尸检证实,活跃的巩膜组织丧失与胶原蛋白有关,其次是黏多糖的消耗[21],但动物模型显示这是一个复杂的过程,涉及巩膜基质的加速降解,以及正视转变为近视后新的细胞外基质的产生减缓[13]。基质降解的过程和原因已经有研究报道,基质金属蛋白酶(MMPs)的产生和激活增加[16,22],以及其调节剂即MMPs的组织抑制剂(TIMPs)的活性降低[23],是关键的促进因素。新的细胞外基质的产生减少表现为胶原蛋白的合成水平降低,特别是I型胶原蛋白[13],以及蛋白聚糖、糖胺聚糖(GAG)侧链的合成减少[8]。但是这是一个复杂的过程,因为它们在新基质的分子成分之间形成明显的计量不平衡,导致有可能在形态学层面观察到的纤维形态和基质结构的差异。特别是有证据表明,细胞外基质成分合成的广泛减少将影响许多参与胶原蛋白纤维直径的关键因素,如V型胶原蛋白[13]和较小的蛋白聚糖[24],并可能使较小的胶原蛋白纤维比例更高。巩膜基质的多糖表达谱会发生明显变化,其中如糖胺聚糖[25]的积累减少,会导致巩膜水化在近视眼中减少。总的来说,上述变化表明,随着近视的发展,巩膜基质成为一种更薄、更不脆弱的生物材料,也越来越容易受到体内生理力量的影响。
巩膜成纤维细胞和肌成纤维细胞是近视眼巩膜细胞外基质变化的协调者,据推测,成纤维细胞转分化是对视网膜局部发出的生化信号的响应,是对投射到视网膜光感受器上并在视网膜层中处理的图像所包含特定信息的响应[26]。然而,在动物模型中的研究表明,在巩膜细胞周围的基质发生变化时,对巩膜细胞也有二次影响,从而导致调控细胞行为的局部应力发生改变。具体而言,体外研究表明巩膜在细胞外基质被削弱的情况下,成纤维细胞受到的压力增加,再加上基质刺激细胞因子(如TGF-β)水平降低,最终导致转分化为肌成纤维细胞的细胞数量增加[27]。尽管有证据表明细胞似乎通过减少整合素的产生而减少与细胞外基质的粘附程度[28],上述结果可能反映了调节细胞局部压力水平和维持周围基质完整性间存在某种平衡。
鉴于近视眼巩膜发生的结构和生化变化以及这些变化的影响,在近视眼的研究中巩膜的机械性能往往特别能引起研究者的兴趣。
近视眼巩膜的生物力学特性
在发现高度近视眼的巩膜会明显变薄后,人们很早就意识到,巩膜的生物力学特性也必将受到影响。随后,对高度近视眼尸检的生物力学特性分析证实了上述情况。研究表明,来自 “正常”正视眼的巩膜往往显示出区域性的硬度变化,如眼球后部的巩膜具有较低的杨氏(弹性)模量,因此硬度低于赤道或前部区域[29],而近视眼中相同区域的巩膜组织显示出更低的硬度值,表明近视眼的巩膜在体内可能更具延展性[30]。
由于巩膜是一种具有粘弹性的生物组织,随后的研究确定了其对模仿眼睛生理负荷曲线作用力的反应特性,指出近视眼的巩膜结构特点使其在长期暴露于眼内压的正常作用力时更容易膨胀[31]。近视眼巩膜组织在生理负荷下发生“蠕动”的趋势表明,近视眼巩膜重塑和超微结构变化的生化因素导致巩膜组织出现生物力学上的弱点,从而随着时间的推移导致眼球伸长和近视发展。为了支持这一假设,进一步的研究表明近视眼的后部巩膜的蠕变特性与该眼的眼球伸长程度和近视发展之间存在关联[31]。
巩膜胶原蛋白的含量对巩膜的生物力学特性有很大影响,其中原纤维直径起着重要作用[12],而巩膜中不同类型胶原蛋白的相对比例也对此有影响[13]。胶原纤维束之间的交联数量也会调节巩膜的生物力学,随着年龄和交联数量的增加,巩膜会变得更硬[32]。蛋白聚糖的浓度在整个生命过程中都会发生变化,而这也可能是与年龄相关的巩膜生物力学特性变化的部分原因[15]。一系列其他的生长因子和信号级联反应参与了巩膜组织的结构维持,从而影响了巩膜的生物力学行为[33]。胶原蛋白和蛋白聚糖概况的变化以及胶原蛋白交联的改变可能是近视发展过程中巩膜生物力学变化的关键因素。
现在,人们普遍认为巩膜的生物力学特性与其超微结构和生物化学组成密切相关,巩膜生物化学的特定变化会促进眼球增大和近视。因此,阻止眼球过度增长的措施,要么是加强巩膜的细胞外基质,要么是逆转驱动近视眼中巩膜机械性弱点的生物化学变化。
近视眼巩膜的强化方法
人们已经采用了许多策略来加强巩膜以防止近视的发展,每种策略都针对控制巩膜生物力学特性因素的不同方面。
后巩膜加固术(PSR)
PSR手术由Shevelev于1930年首次提出,在高度近视的治疗中有零星的使用历史[34]。PSR的目的是作为一个带扣,改善近视巩膜的生物力学特性。由于该手术的高度侵入性,它通常只在病理性近视程度最高的患者中进行,这类患者经常有葡萄肿形成和(或)近视性黄斑脱离。多年来,使用供体组织或合成材料开发的各种PSR技术都取得了不同程度的成功。PSR手术近期的支持者主要是来自俄罗斯和中国。早期PSR手术的生物力学缺陷被证实是对眼球球体后方施力过小的结果,而应用适当张力的巩膜扣能有效控制轴向近视的发展[35]。使用人类供体巩膜[6,36]和硬脑膜[37]作为加固材料,可以看到轴向长度和屈光进展的远期减少。然而,在年轻的高度近视患者中,仅能观察到PSR的轻微效果[38]。在所有情况下,PSR仅用于控制高度、病理性近视,而不是控制与较低水平(生理性)近视的过度轴向伸长。
尽管PSR通常用于病理性近视,但PSR导致巩膜发生的解剖学及生物力学变化也可能为较低水平(生理性)近视的发展提供启示。较少研究关注组织对PSR的反应,而且迄今为止只在动物模型中进行了研究。在兔巩膜中使用合成加固材料会导致炎症性伤口愈合反应和III型胶原蛋白肉芽组织沉积,最终被I型胶原蛋白取代[39]。当人类供体巩膜被用来加固兔子巩膜时,也会出现类似的炎症反应,继而是纤维化[40]。PSR手术后,巩膜的弹性模量和羟脯氨酸含量在加固的巩膜区域都有所下降,但在9个月左右恢复到生理水平[40]。由此看来,加固的时间越长,发生的巩膜弹性模量增加越大,而且这种巩膜弹性模量的增加与加固材料自身的模量没有直接关系[41]。与对照组织相比,加固区域的巩膜成纤维细胞对机械刺激的反应也有所改变[42-44]。PSR手术后,术眼巩膜中单个巩膜成纤维细胞的粘弹性降低,但受体和供体组织之间融合区的成纤维细胞的粘弹性明显增加[42]。与正常对照组相比,PSR导致受体组织中的MMP-2减少,其中受体和供体组织之间的过渡区减少最为明显[44]。成纤维细胞的循环拉伸可以进一步降低MMP-2的水平[45]。通过机械拉伸,受体和供体组织之间过渡区的TGF-β1和FGF-2水平也明显增加,这表明在机械力作用下PSR区域的融合和增厚会增强。综上所述,PSR手术后巩膜弹性模量的增加是由于供体组织诱导受体组织重塑,与近视发展过程中看到的恰好相反。PSR手术后巩膜反应发生的机制尚未完全阐明,但针对信号级联本身可能同样有用,可以在无需手术干预的情况下影响近视眼的进展。
胶原交联术
虽然PSR手术已经实施了几十年,但最近在加强后巩膜以防止近视发展方面的尝试主要集中在巩膜胶原交联上。在过去的20年里,胶原交联已经成功地用于加强角膜扩张症,特别是圆锥角膜[46]。巩膜中的胶原蛋白自然含有交联,并随年龄增长呈现出类型的变化[14]和交联数量的增加[47]。巩膜硬度随年龄增长而相应增加可能与上述过程有关[32]。当巩膜胶原交联受到抑制时,会显著促进实验性近视的发展[48],这突出了内源性巩膜胶原交联在屈光不正发展中的重要性。
在角膜交联中,典型的过程包括光诱导剂(核黄素)和光源[紫外线A(UVA)]来诱导交联的形成[46]。一些研究已经使用动物模型来探索核黄素和UVA或蓝光交联巩膜的可行性和抑制近视的效果[34]。最近已经成功利用体内的巩膜交联阻止了兔子实验性近视的发展[49],在戴负镜的豚鼠身上也有短期成功的报道[50]。体内交联并没有阻止近视引起的胶原纤维束数量减少,但纤维束更密集,分布更规则,并显现出更偏向较厚的纤维束[50]。对没有诱导近视的眼球进行巩膜交联治疗,会存在对眼球生长的抑制,这也进一步证明了巩膜交联阻碍眼球生长的作用[51]。与近视眼相反,当巩膜交联在没有近视诱导的情况下进行时,巩膜胶原纤维束会变得偏向于较小的直径[52],纤维束组成的可变性也会增加[53]。在生物力学上,交联的巩膜出现弹性和粘性模量的增加[53-55],以及硬度的增加[53]。当人类巩膜在体外交联时,其反应类似于在体内观察到的反应,即偏向于更厚的胶原纤维直径以及纤维直径分布的总体增加[55,56]。人类[57]和兔子巩膜[58]的杨氏模量随着交联光源强度的增加而增加,但是这种效应会趋于平稳,并且平稳后随着强度的进一步增加,杨氏模量会下降[57]。如果照射水平不够,则看不到生物力学效应[59]。
巩膜对不同照射强度的反应各不相同,这使确定给定近视程度和巩膜胶原蛋白含量的合适交联水平成为一个挑战。在大多数情况下,不断增加的照射强度能使巩膜变硬,但不断增加的照射强度会增加并发症的风险。巩膜炎症[58]、胶原蛋白破坏或紊乱[52,53,55]、暗适应视网膜电图(ERG)减少[54]、视网膜细胞死亡和层次破坏[51-54,58]都是在交联手术后观察到的,特别是在较高的照射水平下。考虑到减缓近视发展所需的生物力学改变需要更高的照射强度来实现,因此在开始人体交联试验之前,有必要对当前程序可能出现的严重副作用进行细致和深入的调查。还有研究者对交联过程对巩膜肌成纤维细胞和血管的影响表示担忧[60],如果胶原交联将成为一种可行的治疗策略,那么这些问题也需要得到解决。
巩膜交联的过程中,核黄素和UVA或蓝光需要较长时间才能传递到巩膜表面,这使得这种高度侵入性的手术对于控制普通人群的近视是不现实的。将光传送到巩膜后表面所涉及的一些困难,可能会随着可提高光传送性能的柔性光波导的发展来克服[61],或者可以通过多模光纤以微创的方式传输核黄素和UVA[62]。除了传统交联技术之外可以使用其他交联剂,这些交联剂不需要繁琐的、可能有毒的激活过程就能发挥作用。京尼平和甘油醛都被证明可以在体外增加巩膜的杨氏模量[63,64],在体外也证明了巩膜硬度增加呈现剂量依赖性[65]。京尼平已证明能有效地防止眼轴伸长和近视的发展。在形觉剥夺的豚鼠模型中京尼平促进巩膜杨氏模量的增加,同时伴随着胶原纤维直径的增加和纤维密度的降低[66]。然而,有趣的是,虽然甘油醛如预期一样可以增加巩膜应力和杨氏模量,但是它在防止近视发展方面没有显示出显著效果[64]。其他已被研究的用于加强巩膜胶原交联的药剂包括甲基乙二醛[65]和一系列甲醛释放剂如羟甲基甘氨酸钠[67,68],可能对预防近视发展有益。
虽然这些新形式的巩膜交联在控制近视方面仍处于起步阶段,但重要的是需要考虑到潜在的长期副作用,而不是前面提到的直接的组织学影响。在小鼠模型中用甘油醛进行巩膜胶原交联可使巩膜变硬,但对视网膜组织学或ERG功能没有影响。然而甘油醛可使眼内压增加并导致视网膜神经节细胞的损失加快[69]。虽然这种巩膜交联对青光眼损害的增加可能仅限于使用甘油醛作为药剂,但它也可能与其他形式的巩膜交联预防近视有关。鉴于近视和青光眼之间的流行病学联系,应该将其上述情况考虑在内[70]。
其他物理强化手段
一系列材料的Tenon囊下注射或植入已被批准试用,并作为加固巩膜和防止近视发展的一种手段[71-74]。兔眼的巩膜强化注射可导致肉芽肿性炎症,然后被新的胶原蛋白缓慢取代[71],这反映了合成带巩膜强化的效果[39]。该手术似乎也是减缓人眼进行性高度近视的可行方案,在注射后4-5年内,约有50%的患者能保持稳定的屈光状态[71]。Tenon囊下注射由丙烯酸透明质酸组成的水凝胶也成功阻止了豚鼠实验性近视的进展,但有趣的是,注射仅含有缓冲液的对照眼也显示出抑制近视发展的相似效果[72]。在这些案例中,水凝胶植入物有细胞浸润,植入物和对照注射部位的Tenon囊增厚,表明处于炎症过程的早期阶段。然而在雏鸡中,Tenon囊下的强化注射虽然导致了巩膜增厚,但并没有影响眼睛的生长[73,74],这提示了哺乳动物和禽类眼睛生物力学生长特征中不同的机制作用。
理论上,另一个潜在的近视控制策略是将干细胞输送到巩膜下的空间[75]。有研究假设,植入的干细胞可能被诱导成成纤维细胞以增强巩膜胶原蛋白的生产,或作用于多巴胺能途径以调节近视的发展。为了防止近视发展,人类成纤维细胞已经通过Tenon囊下注射移植到后巩膜的悬浮液中[76]。在移植成纤维细胞的眼睛中,形觉剥夺引起的近视率降低了约40%。,并且植入位置周围的巩膜中I型胶原蛋白增加,而移植四周后的成纤维细胞数量减少。据推测,增加的胶原蛋白沉积加固了巩膜,从而减少近视的发展。
胶原蛋白和蛋白聚糖
加强巩膜的另一种方法是更直接地阻止近视发展过程中出现的组织退化,或加强新组织的产生以抵消退化。
在近视的发展过程中,巩膜中的胶原蛋白含量显著减少,纤维变薄,纤维厚度梯度消失,巩膜厚度也普遍减少[26]。咖啡因的代谢物7-甲基黄嘌呤是一种非选择性腺苷拮抗剂,已被证实可以阻止豚鼠[77]和兔[78]的形觉剥夺性近视发展,但其实际作用机制尚不清楚。它还在控制雏鸡的晶状体诱导性近视方面显示出中度的效果[79],并几乎完全阻止了恒河猴的晶状体诱导性近视的发展[80]。在没有诱导近视发展的眼睛中,7-甲基黄嘌呤明显增加后巩膜胶原纤维的直径,并明显减少GAG总含量[81]。巩膜胶原纤维直径的改变可能是7-甲基黄嘌呤抑制近视的作用机制,因为用它治疗还可以防止近视发展中常见的胶原纤维梯度损失[26,77,78]。7-甲基黄嘌呤在临床试验中也被证明能显著减少眼轴伸长和近视的发展[82,83],而停止治疗后,眼轴伸长恢复到与对照组相同的速率[84]。超过8年的长期随访显示,口服7-甲基黄嘌呤治疗后近视发展可减少约60%[85]。胶原蛋白合成途径中的其他分子,包括过氧化物酶体增殖剂激活受体[80,86]、环腺苷单磷酸酯[87]、环鸟苷单磷酸酯[88]、G蛋白信号调节器2[89]和骨形态发生蛋白(BMP)[90-92],也具有增加巩膜胶原蛋白含量和加强巩膜以阻碍近视进展的潜在作用。
相反,与其增加巩膜胶原蛋白的产生来强化巩膜,不如通过调节MMPs和TIMPs来减缓原有胶原蛋白的降解。补充TIMP-2能显著减少雏鸡[5]和树鼩[23]近视发展过程中的胶原蛋白降解量,尽管仅在树鼩中观察到近视量的相应减少。哌仑西平是一种可有效控制人类近视的发展[93]的M1受体选择性抗毒蕈碱剂,并且可通过调节MMP-2和TIMP-2的表达来抑制实验性近视的发展[94]。从山桑子中提取的花青素可通过抑制MMP-2和增强I型胶原蛋白的表达来减少形式剥夺的近视[95]。从黑加仑中提取的花青素也被证明可以防止晶状体诱导性和形觉剥夺性近视[96,97],尽管其作用机制尚未明确,但它们可能是通过相似的途径发挥作用。Sonic hedgehog(Shh)通路已被证明参与并促进了小鼠和豚鼠的近视发展,而Shh通路的抑制剂环丙胺能阻止近视发展[98,99]。Shh通路蛋白的表达在近视诱导过程中增加,并与蓝色及红色视蛋白的视网膜mRNA表达增加相一致,表明Shh通路在屈光发育过程中是视蛋白的调节上游[100]。然而,Shh通路促进近视的发展似乎是通过增加MMP-2的产生来实现的[99],这为控制近视提供了另一个潜在靶点。
GAG含量在近视眼巩膜中的减少与近视发展相关的巩膜蠕变峰值的增加有关[101]。然而,去除巩膜中的硫酸化GAG会不同程度地增加人类的巩膜硬度[102],并降低猪的巩膜硬度[103]。BMP-2[91]和胰岛素[104]可以上调巩膜GAG的合成。巩膜成纤维细胞中的GAG水平在GABA激动剂的作用下会降低,而在GABA拮抗剂的作用下会升高[105],这可能是涉及巩膜内测和巩膜外侧GABA受体的双重机制。有趣的是,阿托品是迄今为止发现的抑制人类近视发展最成功的治疗方法之一[106],它能显著逆转近视发展过程中视网膜和巩膜GABA转运蛋白1水平的升高[107]。因此,阿托品的近视预防作用至少部分是通过减少GABA介导的GAG抑制作用而实现的。过氧化物酶体激活剂受体α(PPRAα)最近被证明可以通过增加巩膜胶原的合成来抑制近视的发展[80],也被证明可以抑制GAG的生物合成并抵消TGF-β1对其他组织中蛋白聚糖合成的刺激作用[108]。β-木糖苷对蛋白聚糖合成的抑制可以阻止雏鸡形觉剥夺性近视的发生[109]。蛋白聚糖及其GAG侧链在近视发展中的作用显然是非常复杂的,这代表了不同核心蛋白之间表达水平的复杂平衡以及结合的GAG侧链组成。操纵蛋白聚糖和GAG来控制巩膜生物力学从而控制近视的发展,虽然在理论上很有前景,但到目前为止成功率很低。
成纤维细胞、肌成纤维细胞和 TGF-β
巩膜肌成纤维细胞是由于α-SMA的存在而产生具有收缩特性的成纤维细胞,其存在于人类[18]、猕猴[18]、树鼩[110]和豚鼠[111]巩膜中。这些细胞的收缩特性首次在伤口愈合的肉芽组织中被发现[113],并成为了阻止甚至可能逆转近视发展的一个有趣靶标。为了使成纤维细胞转分化为肌成纤维细胞表型并表达α-SMA,它们必须同时暴露于TGF-β[114]和局部组织应激[115]。
TGF-β的表达在近视眼中是下调的,并导致胶原蛋白合成的减少[17]。然而,在巩膜所受压力不变的情况下,TGF-β的减少实际上增加了培养的巩膜成纤维细胞/肌成纤维细胞中α-SMA的表达,这可能是通过减少胶原合成和增加细胞局部压力来实现的[27]。上述结果意味着在控制近视的过程中,针对肌成纤维细胞存在多种激活途径。TGF-β参与近视进展中巩膜变化的一个途径是Wnt/β-catenin信号通路。最近有研究表明,拮抗剂DKK-1在实验性近视中抑制Wnt/β-catenin通路会增加I型胶原蛋白的表达,并诱导胶原蛋白更有序地排列,而中和TGF-β1则能够进一步减少I型胶原蛋白的表达[116]。此外,即使在没有近视刺激的情况下,激活Wnt途径也会导致屈光状态的近视转变[117]。传统中药补精益施方能以剂量依赖的方式延缓形觉剥夺性近视的发展,增加巩膜TGF-β1和Smad3水平,并导致巩膜厚度和成纤维细胞数量的增加[118]。Smad3是协助TGF-β信号从成纤维细胞外转移到细胞核的效应蛋白家族之一,也是激活α-SMA和肌成纤维细胞分化的潜在途径之一[114]。有趣的是Wnt/β-catenin通路似乎也在激活α-SMA的途径中起着关键作用[114]。
环孢素A是一种免疫调节药物,可增加某些组织中成纤维细胞的TGF-β、α-SMA和I型胶原蛋白的表达[119,120],同时在某些组织类型中具有抑制作用[121,122]。有趣的是,最近已经证实环孢素A可以延缓实验性近视的发展,但这是否是通过调节α-SMA的表达来实现的尚无定论[123]。在牙龈成纤维细胞中,环孢素A通过Shh通路增强了α-SMA和I型胶原蛋白的表达,并受到了TGF-β的调节[119]。阿托品也被证明可以减少巩膜中的炎症标志物,同时抑制近视的发展[123]。上述结果提示了近视发展中与炎症存在联系的可能性。事实上,关于近视发病机制的早期理论之一——近视是由视网膜、脉络膜和巩膜的低度炎症引起的:”炎症通常是在视网膜和脉络膜充血之前发生,而且是过度和不适当使用眼睛的结果。炎症会缓慢加重,但不会变得活跃。儿童成长过程中的近视无疑是这个原因造成的”[124]。最近,有研究发现近视在患有系统性、炎症性疾病的人群中发生率更高[123],并呈现出与花粉热的相关性[125],佩戴负镜引起的近视与补体因子的增加有关[126]。近视和其他炎症性眼病之间也有联系[127]。通过差异基因表达分析,已经证实免疫途径也在屈光发展中发挥作用[128]。
巩膜中细胞活动可能是在控制近视眼巩膜生物力学变化中发挥作用的另一个方面,是成纤维细胞和肌成纤维细胞对周围细胞外基质的粘附。介导细胞-基质相互作用的整合素在哺乳动物巩膜中表达[19,129],并在近视发展过程中出现明显的表达下调[28,130]。碱性成纤维细胞生长因子可以增加巩膜中整合素和I型胶原蛋白的表达,同时抑制近视的发展[130]。
显然,成纤维细胞和肌成纤维细胞在巩膜变化和近视发展中的相互作用十分复杂,其中涉及许多不同的生长因子和信号通路。虽然这使人们确定近视发病机制的途径变得更加困难,但它确实为加强巩膜和延缓近视发展提供了许多潜在的靶点,未来也需要深入进行研究。
候选基因
已有大量的研究关注近视发展过程中的基因表达模式,对参与近视发展的基因进行全面描述已经超出了本综述的范围。分析表明,参与人类近视发展的候选基因与近视实验动物模型中鉴定的候选基因呈现明显的重叠,表明这些模型仍然适合研究人类近视发展[131]。大量基因在近视的发展或恢复过程中上调或下调,其中一些基因是双向的。由于存在大量的候选基因,仍然不清楚哪些基因应该进行深入探索以了解它们在近视发展中的作用,尤其是它们在巩膜中的作用。事实上,这种情况很可能反映了基因的微小表达变化和之间的相互作用从而导致了近视表型[132],当然,巩膜中的许多表型变化发生在一些候选基因的信号级联下游。最近一些研究指出miRNA,即调节基因表达的小型非编码RNAs,可能参与近视的发展[133-136]。随着大量非病理性近视的候选基因通过测序被鉴定出,控制近视发展的潜在巩膜靶标有待进一步研究。
巩膜信号传递
本综述尚未涉及的一个方面是近视发展过程中发生的变化是如何传递到巩膜的。大量研究表明,眼球生长存在局部控制,视网膜能检测到离焦信号并作出反应[137]。有证据表明,上述过程至少部分受到脉络膜厚度变化的调节,脉络膜释放的介质可能向巩膜发出信号并改变其生长参数[138]。有许多潜在的视网膜-脉络膜信号的候选者,对这些信号的讨论不在本综述的范围内,但是需要说明的是这些信号如果被成功鉴定出来,将提供上游靶标用于调节近视发展过程中巩膜的生物化学行为和生物力学行为。
总结
在近视发展过程中,巩膜发生的变化代表了组织重塑、合成和降解之间复杂的相互作用,其结果是巩膜硬度降低和后巩膜的可伸展性增加。人们已经采用了许多不同的策略来加固巩膜以防止近视的发展,这些策略大致可分为加强被削弱的巩膜组织,或调节合成和降解途径。迄今为止,在人类身上采用的最成功的技术是后巩膜加固术,但由于该技术的高度侵入性和不稳定的结果,使其通常仅被用于最严重的病理性近视。巩膜胶原蛋白交联术代表了一种有前景的PSR替代方法。与在治疗角膜扩张症方面备受青睐的角膜交联术不同,巩膜交联术由于需要进入后巩膜,且靠近视网膜和巩膜血管等对紫外线损伤敏感的组织,因此目前仍很难实施。7-甲基黄嘌呤对胶原蛋白合成的调节和对MMP-2的抑制显示出加强巩膜组织的前景,但是其针对其他途径的结果却喜忧参半。
因此,加强巩膜以防止近视发展所面临的挑战主要有两个方面。首先是后巩膜在眼眶中的位置,其次是我们尚未完全阐明的巩膜重塑中众多精细平衡过程。我们目前对巩膜生物化学和生物力学过程的理解表明,本应该加强巩膜的干预措施并没有发挥预期的作用,因此这一事实也提示我们,开发一种可行的、功能性的巩膜稳定技术来预防近视,这将取决于我们对一系列复杂的组织相互作用的理解。
Acknowledgments
Funding: None.
Footnote
Provenance and Peer Review: This article was commissioned by the Guest Editors (Ian G. Morgan, Xiaohu Ding and Xinxing Guo) for the series “Managing Myopia in East Asia Myopia Crisis” published in Annals of Eye Science. The article has undergone external peer review.
Conflicts of Interest: Both authors have completed the ICMJE uniform disclosure form (available at http://dx.doi.org/10.21037/aes.2018.01.04). The series “Managing Myopia in East Asia Myopia Crisis” was commissioned by the editorial office without any funding or sponsorship. The authors have no other conflicts of interest to declare.
Ethical Statement: The authors are accountable for all aspects of the work in ensuring that questions related to the accuracy or integrity of any part of the work are appropriately investigated and resolved.
Open Access Statement: This is an Open Access article distributed in accordance with the Creative Commons Attribution-NonCommercial-NoDerivs 4.0 International License (CC BY-NC-ND 4.0), which permits the non-commercial replication and distribution of the article with the strict proviso that no changes or edits are made and the original work is properly cited (including links to both the formal publication through the relevant DOI and the license). See: https://creativecommons.org/licenses/by-nc-nd/4.0/.
References
- Zadnik K. The Glenn A. Fry Award Lecture (1995). Myopia development in childhood. Optom Vis Sci 1997;74:603-8. [Crossref] [PubMed]
- Javitt JC, Chiang YP. The socioeconomic aspects of laser refractive surgery. Arch Ophthalmol 1994;112:1526-30. [Crossref] [PubMed]
- Meskauskas J, Repetto R, Siggers JH. Shape change of the vitreous chamber influences retinal detachment and reattachment processes: is mechanical stress during eye rotations a factor? Invest Ophthalmol Vis Sci 2012;53:6271-81. [Crossref] [PubMed]
- Curtin BJ, Teng CC. Scleral changes in pathological myopia. Trans Am Acad Ophthalmol Otolaryngol 1958;62:777-88; discussion 88-90. [PubMed]
- Liu HH, Gentle A, Jobling AI, et al. Inhibition of matrix metalloproteinase activity in the chick sclera and its effect on myopia development. Invest Ophthalmol Vis Sci 2010;51:2865-71. [Crossref] [PubMed]
- Li XJ, Yang XP, Li QM, et al. Posterior scleral reinforcement for the treatment of pathological myopia. Int J Ophthalmol 2016;9:580-4. [PubMed]
- Norton TT, Siegwart JT Jr. Animal models of emmetropization: matching axial length to the focal plane. J Am Optom Assoc 1995;66:405-14. [PubMed]
- McBrien NA, Lawlor P, Gentle A. Scleral remodeling during the development of and recovery from axial myopia in the tree shrew. Invest Ophthalmol Vis Sci 2000;41:3713-9. [PubMed]
- Bron A, Tripathi R, Tripathi B. The cornea and sclera. Wolff's Anatomy Of The Eye And Orbit. 8th Edition. London: Chapman and Hall Medical, 1997:233-78.
- Komai Y, Ushiki T. The three-dimensional organization of collagen fibrils in the human cornea and sclera. Invest Ophthalmol Vis Sci 1991;32:2244-58. [PubMed]
- Park CY, Marando CM, Liao JA, et al. Details of the collagen and elastin architecture in the human limbal conjunctiva, tenon's capsule and sclera revealed by two-photon excited fluorescence microscopy. Invest Ophthalmol Vis Sci 2016;57:5602-10. [Crossref] [PubMed]
- McBrien NA, Cornell LM, Gentle A. Structural and ultrastructural changes to the sclera in a mammalian model of high myopia. Invest Ophthalmol Vis Sci 2001;42:2179-87. [PubMed]
- Gentle A, Liu Y, Martin JE, et al. Collagen gene expression and the altered accumulation of scleral collagen during the development of high myopia. J Biol Chem 2003;278:16587-94. [Crossref] [PubMed]
- Bailey AJ. Structure, function and ageing of the collagens of the eye. Eye (Lond) 1987;1:175-83. [Crossref] [PubMed]
- Rada JA, Achen VR, Penugonda S, et al. Proteoglycan composition in the human sclera during growth and aging. Invest Ophthalmol Vis Sci 2000;41:1639-48. [PubMed]
- Siegwart JT Jr, Norton TT. Selective regulation of MMP and TIMP mRNA levels in tree shrew sclera during minus lens compensation and recovery. Invest Ophthalmol Vis Sci 2005;46:3484-92. [Crossref] [PubMed]
- Jobling AI, Nguyen M, Gentle A, et al. Isoform-specific changes in scleral transforming growth factor-beta expression and the regulation of collagen synthesis during myopia progression. J Biol Chem 2004;279:18121-6. [Crossref] [PubMed]
- Poukens V, Glasgow BJ, Demer JL. Nonvascular contractile cells in sclera and choroid of humans and monkeys. Invest Ophthalmol Vis Sci 1998;39:1765-74. [PubMed]
- Metlapally R, Jobling AI, Gentle A, et al. Characterization of the integrin receptor subunit profile in the mammalian sclera. Mol Vis 2006;12:725-34. [PubMed]
- Hayashi M, Ito Y, Takahashi A, et al. Scleral thickness in highly myopic eyes measured by enhanced depth imaging optical coherence tomography. Eye (Lond) 2013;27:410-7. [Crossref] [PubMed]
- Avetisov ES, Savitskaya NF, Vinetskaya MI, et al. A study of biochemical and biomechanical qualities of normal and myopic eye sclera in humans of different age groups. Metab Pediatr Syst Ophthalmol 1983;7:183-8. [PubMed]
- Guggenheim JA, McBrien NA. Form-deprivation myopia induces activation of scleral matrix metalloproteinase-2 in tree shrew. Invest Ophthalmol Vis Sci 1996;37:1380-95. [PubMed]
- Liu HH, Kenning MS, Jobling AI, et al. Reduced scleral TIMP-2 expression is associated with myopia development: TIMP-2 supplementation stabilizes scleral biomarkers of myopia and limits myopia development. Invest Ophthalmol Vis Sci 2017;58:1971-81. [Crossref] [PubMed]
- Rada JA, Nickla DL, Troilo D. Decreased proteoglycan synthesis associated with form deprivation myopia in mature primate eyes. Invest Ophthalmol Vis Sci 2000;41:2050-8. [PubMed]
- Moring AG, Baker JR, Norton TT. Modulation of glycosaminoglycan levels in tree shrew sclera during lens-induced myopia development and recovery. Invest Ophthalmol Vis Sci 2007;48:2947-56. [Crossref] [PubMed]
- McBrien NA, Gentle A. Role of the sclera in the development and pathological complications of myopia. Prog Retin Eye Res 2003;22:307-38. [Crossref] [PubMed]
- Jobling AI, Gentle A, Metlapally R, et al. Regulation of scleral cell contraction by transforming growth factor-beta and stress: competing roles in myopic eye growth. J Biol Chem 2009;284:2072-9. [Crossref] [PubMed]
- McBrien NA, Metlapally R, Jobling AI, et al. Expression of collagen-binding integrin receptors in the mammalian sclera and their regulation during the development of myopia. Invest Ophthalmol Vis Sci 2006;47:4674-82. [Crossref] [PubMed]
- Friberg TR, Lace JW. A comparison of the elastic properties of human choroid and sclera. Exp Eye Res 1988;47:429-36. [Crossref] [PubMed]
- Curtin BJ. Physiopathologic aspects of scleral stress-strain. Trans Am Ophthalmol Soc 1969;67:417-61. [PubMed]
- Phillips JR, Khalaj M, McBrien NA. Induced myopia associated with increased scleral creep in chick and tree shrew eyes. Invest Ophthalmol Vis Sci 2000;41:2028-34. [PubMed]
- Schultz DS, Lotz JC, Lee SM, et al. Structural factors that mediate scleral stiffness. Invest Ophthalmol Vis Sci 2008;49:4232-6. [Crossref] [PubMed]
- Harper AR, Summers JA. The dynamic sclera: extracellular matrix remodeling in normal ocular growth and myopia development. Exp Eye Res 2015;133:100-11. [Crossref] [PubMed]
- Ohno-Matsui K. Sclera-targeted therapies for pathologic myopia. In: Spaide R, Ohno-Matsui K, Yannuzzi L. editors. Pathologic Myopia. New York, NY: Springer 2014:353-60.
- Ward B, Tarutta EP, Mayer MJ. The efficacy and safety of posterior pole buckles in the control of progressive high myopia. Eye (Lond) 2009;23:2169-74. [Crossref] [PubMed]
- Shen ZM, Zhang ZY, Zhang LY, et al. Posterior scleral reinforcement combined with patching therapy for pre-school children with unilateral high myopia. Graefes Arch Clin Exp Ophthalmol 2015;253:1391-5. [Crossref] [PubMed]
- Chen M, Dai J, Chu R, et al. The efficacy and safety of modified Snyder-Thompson posterior scleral reinforcement in extensive high myopia of Chinese children. Graefes Arch Clin Exp Ophthalmol 2013;251:2633-8. [Crossref] [PubMed]
- Xue A, Bao F, Zheng L, et al. Posterior scleral reinforcement on progressive high myopic young patients. Optom Vis Sci 2014;91:412-8. [Crossref] [PubMed]
- Jacob JT, Gebhardt BM, Lewando J. Synthetic scleral reinforcement materials. II. Collagen types in the fibrous capsule. J Biomed Mater Res 1996;32:181-6. [Crossref] [PubMed]
- Weiyi C, Wang X, Wang C, et al. An experimental study on collagen content and biomechanical properties of sclera after posterior sclera reinforcement. Clin Biomech (Bristol, Avon) 2008;23:S17-20. [Crossref] [PubMed]
- Yan Z, Wang C, Chen W, et al. Biomechanical considerations: evaluating scleral reinforcement materials for pathological myopia. Can J Ophthalmol 2010;45:252-5. [Crossref] [PubMed]
- Wang G, Chen W. Effects of mechanical stimulation on viscoelasticity of rabbit scleral fibroblasts after posterior scleral reinforcement. Exp Biol Med (Maywood) 2012;237:1150-4. [Crossref] [PubMed]
- Wang G, Hao S, Deng A. Effects of mechanical stimulation on TGF-β1 and bFGF expression of scleral fibroblasts after posterior sclera reinforcement. 2013 Icme International Conference on Complex Medical Engineering (Cme); 2013:399-402.
- Wang G, Xie Y, Chen W. The MMP-2 and TIMP-2 expression of sclera and scleral fibroblasts after posterior sclera reinforcement surgery. 2010 3rd International Conference on Biomedical Engineering and Informatics;16-18 Oct 2010.
- Wang GH, Chen WY. Effects of mechanical stimulation on MMP-2 and TIMP-2 expression of scleral fibroblasts after posterior sclera reinforcement. Appl Mech Mater 2014;490-491:867. [Crossref]
- Randleman JB, Khandelwal SS, Hafezi F. Corneal cross-linking. Surv Ophthalmol 2015;60:509-23. [Crossref] [PubMed]
- Malik NS, Moss SJ, Ahmed N, et al. Ageing of the human corneal stroma: structural and biochemical changes. Biochim Biophys Acta 1992;1138:222-8. [Crossref] [PubMed]
- McBrien NA, Norton TT. Prevention of collagen crosslinking increases form-deprivation myopia in tree shrew. Exp Eye Res 1994;59:475-86. [Crossref] [PubMed]
- Dotan A, Kremer I, Livnat T, et al. Scleral cross-linking using riboflavin and ultraviolet-a radiation for prevention of progressive myopia in a rabbit model. Exp Eye Res 2014;127:190-5. [Crossref] [PubMed]
- Liu S, Li S, Wang B, et al. Scleral cross-linking using riboflavin UVA irradiation for the prevention of myopia progression in a guinea pig model: blocked axial extension and altered scleral microstructure. PLoS One 2016;11:e0165792 [Crossref] [PubMed]
- Iseli HP, Korber N, Koch C, et al. Scleral cross-linking by riboflavin and blue light application in young rabbits: damage threshold and eye growth inhibition. Graefes Arch Clin Exp Ophthalmol 2016;254:109-22. [Crossref] [PubMed]
- Karl A, Makarov FN, Koch C, et al. The ultrastructure of rabbit sclera after scleral crosslinking with riboflavin and blue light of different intensities. Graefes Arch Clin Exp Ophthalmol 2016;254:1567-77. [Crossref] [PubMed]
- Iseli HP, Korber N, Karl A, et al. Damage threshold in adult rabbit eyes after scleral cross-linking by riboflavin/blue light application. Exp Eye Res 2015;139:37-47. [Crossref] [PubMed]
- Wang M, Zhang F, Liu K, et al. Safety evaluation of rabbit eyes on scleral collagen cross-linking by riboflavin and ultraviolet A. Clin Exp Ophthalmol 2015;43:156-63. [Crossref] [PubMed]
- Schuldt C, Karl A, Korber N, et al. Dose-dependent collagen cross-linking of rabbit scleral tissue by blue light and riboflavin treatment probed by dynamic shear rheology. Acta Ophthalmol 2015;93:e328-36. [Crossref] [PubMed]
- Choi S, Lee SC, Lee HJ, et al. Structural response of human corneal and scleral tissues to collagen cross-linking treatment with riboflavin and ultraviolet A light. Lasers Med Sci 2013;28:1289-96. [Crossref] [PubMed]
- Zhang M, Zou Y, Zhang F, et al. Efficacy of blue-light cross-linking on human scleral reinforcement. Optom Vis Sci 2015;92:873-8. [Crossref] [PubMed]
- Zhang Y, Zou C, Liu L, et al. Effect of irradiation time on riboflavin-ultraviolet-A collagen crosslinking in rabbit sclera. J Cataract Refract Surg 2013;39:1184-9. [Crossref] [PubMed]
- Zhang Y, Li Z, Liu L, et al. Comparison of riboflavin/ultraviolet-A cross-linking in porcine, rabbit, and human sclera. Biomed Res Int 2014;2014:194204.
- Elsheikh A, Phillips JR. Is scleral cross-linking a feasible treatment for myopia control? Ophthalmic Physiol Opt 2013;33:385-9. [Crossref] [PubMed]
- Kwok SJJ, Kim M, Lin HH, et al. Flexible optical waveguides for uniform periscleral cross-linking. Invest Ophthalmol Vis Sci 2017;58:2596-602. [Crossref] [PubMed]
- Iomdina EN, Tarutta EP, Semchishen VA, et al. Experimental realization of minimally invasive techniques of scleral collagen cross-linking. Vestn Oftalmol 2016;132:49-58. [Crossref] [PubMed]
- Liu TX, Wang Z. Biomechanics of sclera crosslinked using genipin in rabbit. Int J Ophthalmol 2017;10:355-60. [PubMed]
- Chu Y, Cheng Z, Liu J, et al. The effects of scleral collagen cross-linking using glyceraldehyde on the progression of form-deprived myopia in guinea pigs. J Ophthalmol 2016;2016:3526153.
- Campbell IC, Hannon BG, Read AT, et al. Quantification of the efficacy of collagen cross-linking agents to induce stiffening of rat sclera. J R Soc Interface 2017;14:20170014 [Crossref] [PubMed]
- Wang M, Corpuz CC. Effects of scleral cross-linking using genipin on the process of form-deprivation myopia in the guinea pig: a randomized controlled experimental study. BMC Ophthalmol 2015;15:89. [Crossref] [PubMed]
- Babar N, Kim M, Cao K, et al. Cosmetic preservatives as therapeutic corneal and scleral tissue cross-linking agents. Invest Ophthalmol Vis Sci 2015;56:1274-82. [Crossref] [PubMed]
- Zyablitskaya M, Takaoka A, Munteanu EL, et al. Evaluation of therapeutic tissue crosslinking (TXL) for myopia using second harmonic generation signal microscopy in rabbit sclera. Invest Ophthalmol Vis Sci 2017;58:21-9. [Crossref] [PubMed]
- Kimball EC, Nguyen C, Steinhart MR, et al. Experimental scleral cross-linking increases glaucoma damage in a mouse model. Exp Eye Res 2014;128:129-40. [Crossref] [PubMed]
- Marcus MW, de Vries MM, Junoy Montolio FG, et al. Myopia as a risk factor for open-angle glaucoma: a systematic review and meta-analysis. Ophthalmology 2011;118:1989-94.e2. [Crossref] [PubMed]
- Avetisov ES, Tarutta EP, Iomdina EN, et al. Nonsurgical and surgical methods of sclera reinforcement in progressive myopia. Acta Ophthalmol Scand 1997;75:618-23. [Crossref] [PubMed]
- Garcia MB, Jha AK, Healy KE, et al. A bioengineering approach to myopia control tested in a guinea pig model. Invest Ophthalmol Vis Sci 2017;58:1875-86. [Crossref] [PubMed]
- Su J, Iomdina E, Tarutta E, et al. Effects of poly(2-hydroxyethyl methacrylate) and poly(vinyl-pyrrolidone) hydrogel implants on myopic and normal chick sclera. Exp Eye Res 2009;88:445-57. [Crossref] [PubMed]
- Su J, Wall ST, Healy KE, et al. Scleral reinforcement through host tissue integration with biomimetic enzymatically degradable semi-interpenetrating polymer network. Tissue Eng Part A 2010;16:905-16. [Crossref] [PubMed]
- Janowski M, Bulte JW, Handa JT, et al. Concise review: using stem cells to prevent the progression of myopia-a concept. Stem Cells 2015;33:2104-13. [Crossref] [PubMed]
- Shinohara K, Yoshida T, Liu H, et al. Establishment of novel therapy to reduce progression of myopia in rats with experimental myopia by fibroblast transplantation on sclera. J Tissue Eng Regen Med 2017; [Epub ahead of print]. [Crossref] [PubMed]
- Cui D, Trier K, Zeng J, et al. Effects of 7-methylxanthine on the sclera in form deprivation myopia in guinea pigs. Acta Ophthalmol 2011;89:328-34. [Crossref] [PubMed]
- Nie HH, Huo LJ, Yang X, et al. Effects of 7-methylxanthine on form-deprivation myopia in pigmented rabbits. Int J Ophthalmol 2012;5:133-7. [PubMed]
- Wang K, Nava D, Trier K, et al. Influence of oral 7-methylxanthine on lens-induced and form deprivation myopia in chickens. Invest Ophthalmol Vis Sci 2014;55:3040.
- Smith EL, Arumugam B, Hung LF, et al. The effects of the adenosine antagonist, 7-methylxanthine, on refractive development in rhesus monkeys. In: Benavente-Perez A, Nour A, Ansel T, et al. Abstracts from the 15th International Myopia Conference. Eye and Vision;2016;3:O28.
- Trier K, Olsen EB, Kobayashi T, et al. Biochemical and ultrastructural changes in rabbit sclera after treatment with 7-methylxanthine, theobromine, acetazolamide, or L-ornithine. Br J Ophthalmol 1999;83:1370-5. [Crossref] [PubMed]
- Trier K, Ribel-madsen SM. Axial growth and myopia progression with 7-methylxanthine. A double blind, parallel, placebo controlled clinical trial. Ophthal Physiol Opt 2006;26:40.
- Trier K, Ribel-Madsen SM. Effect of 7-methylxanthine on axial eye growth in myopic children - 24 months follow-up. Invest Ophthalmol Vis Sci 2007;48:4421. [PubMed]
- Trier K, Munk Ribel-Madsen S, Cui D, et al. Systemic 7-methylxanthine in retarding axial eye growth and myopia progression: a 36-month pilot study. J Ocul Biol Dis Infor 2008;1:85-93. [Crossref] [PubMed]
- Trier K. 5. 7-methylxanthine treatment. Acta Ophthalmologica 2015;93:
- Bertrand E, Fritsch C, Diether S, et al. Identification of apolipoprotein A-I as a "STOP" signal for myopia. Mol Cell Proteomics 2006;5:2158-66. [Crossref] [PubMed]
- Tao Y, Pan M, Liu S, et al. cAMP level modulates scleral collagen remodeling, a critical step in the development of myopia. PLoS One 2013;8:e71441 [Crossref] [PubMed]
- Fang F, Pan M, Yan T, et al. The role of cGMP in ocular growth and the development of form-deprivation myopia in guinea pigs. Invest Ophthalmol Vis Sci 2013;54:7887-902. [Crossref] [PubMed]
- Zou L, Liu R, Zhang X, et al. Upregulation of regulator of G-protein signaling 2 in the sclera of a form deprivation myopic animal model. Mol Vis 2014;20:977-87. [PubMed]
- Wang Q, Zhao G, Xing S, et al. Role of bone morphogenetic proteins in form-deprivation myopia sclera. Mol Vis 2011;17:647-57. [PubMed]
- Li H, Cui D, Zhao F, et al. BMP-2 is involved in scleral remodeling in myopia development. PLoS One 2015;10:e0125219 [Crossref] [PubMed]
- Wang Q, Xue ML, Zhao GQ, et al. Form-deprivation myopia induces decreased expression of bone morphogenetic protein-2, 5 in guinea pig sclera. Int J Ophthalmol 2015;8:39-45. [PubMed]
- Huang J, Wen D, Wang Q, et al. Efficacy comparison of 16 interventions for myopia control in children: a network meta-analysis. Ophthalmology 2016;123:697-708. [Crossref] [PubMed]
- Qian L, Zhao H, Li X, et al. Pirenzepine inhibits myopia in guinea pig model by regulating the balance of MMP-2 and TIMP-2 expression and increased tyrosine hydroxylase levels. Cell Biochem Biophys 2015;71:1373-8. [Crossref] [PubMed]
- Deng HW, Tian Y, Zhou XJ, et al. Effect of bilberry extract on development of form-deprivation myopia in the guinea pig. J Ocul Pharmacol Ther 2016;32:196-202. [Crossref] [PubMed]
- Iida H, Nakamura Y, Matsumoto H, et al. Differential effects of black currant anthocyanins on diffuser- or negative lens-induced ocular elongation in chicks. J Ocul Pharmacol Ther 2013;29:604-9. [Crossref] [PubMed]
- Iida H, Nakamura Y, Matsumoto H, et al. Effect of black-currant extract on negative lens-induced ocular growth in chicks. Ophthalmic Res 2010;44:242-50. [Crossref] [PubMed]
- Qian YS, Chu RY, Hu M, et al. Sonic hedgehog expression and its role in form-deprivation myopia in mice. Curr Eye Res 2009;34:623-35. [Crossref] [PubMed]
- Chen M, Qian Y, Dai J, et al. The sonic hedgehog signaling pathway induces myopic development by activating matrix metalloproteinase (MMP)-2 in Guinea pigs. PLoS One 2014;9:e96952 [Crossref] [PubMed]
- Escaño MF, Fujii S, Sekiya Y, et al. Expression of Sonic hedgehog and retinal opsin genes in experimentally-induced myopic chick eyes. Exp Eye Res 2000;71:459-67. [Crossref] [PubMed]
- McBrien NA. Regulation of scleral metabolism in myopia and the role of transforming growth factor-beta. Exp Eye Res 2013;114:128-40. [Crossref] [PubMed]
- Murienne BJ, Chen ML, Quigley HA, et al. The contribution of glycosaminoglycans to the mechanical behaviour of the posterior human sclera. J R Soc Interface 2016;13:20160367 [Crossref] [PubMed]
- Murienne BJ, Jefferys JL, Quigley HA, et al. The effects of glycosaminoglycan degradation on the mechanical behavior of the posterior porcine sclera. Acta Biomater 2015;12:195-206. [Crossref] [PubMed]
- Sheng C, Zhu X, Wallman J. In vitro effects of insulin and RPE on choroidal and scleral components of eye growth in chicks. Exp Eye Res 2013;116:439-48. [Crossref] [PubMed]
- Christian PG, Harkin DG, Schmid KL. GABAergic agents modify the response of chick scleral fibroblasts to myopic and hyperopic eye cup tissues. Curr Eye Res 2014;39:172-87. [Crossref] [PubMed]
- Wolffsohn JS, Calossi A, Cho P, et al. Global trends in myopia management attitudes and strategies in clinical practice. Cont Lens Anterior Eye 2016;39:106-16. [Crossref] [PubMed]
- Barathi VA, Chaurasia SS, Poidinger M, et al. Involvement of GABA transporters in atropine-treated myopic retina as revealed by iTRAQ quantitative proteomics. J Proteome Res 2014;13:4647-58. [Crossref] [PubMed]
- Ghiselli G. Drug-mediated regulation of glycosaminoglycan biosynthesis. Med Res Rev 2017;37:1051-94. [Crossref] [PubMed]
- Rada JA, Johnson JM, Achen VR, et al. Inhibition of scleral proteoglycan synthesis blocks deprivation-induced axial elongation in chicks. Exp Eye Res 2002;74:205-15. [Crossref] [PubMed]
- Phillips JR, McBrien NA. Pressure-induced changes in axial eye length of chick and tree shrew: significance of myofibroblasts in the sclera. Invest Ophthalmol Vis Sci 2004;45:758-63. [Crossref] [PubMed]
- Backhouse S, Phillips JR. Effect of induced myopia on scleral myofibroblasts and in vivo ocular biomechanical compliance in the guinea pig. Invest Ophthalmol Vis Sci 2010;51:6162-71. [Crossref] [PubMed]
- Gabbiani G, Ryan GB, Majno G. Presence of modified fibroblasts in granulation tissue and their possible role in wound contraction. Experientia 1971;27:549. [Crossref] [PubMed]
- Bochaton-Piallat ML, Gabbiani G, Hinz B. The myofibroblast in wound healing and fibrosis: answered and unanswered questions. F1000Res 2016;5. pii: F1000 Faculty Rev-752.
- Carthy JM. TGFbeta signaling and the control of myofibroblast differentiation: Implications for chronic inflammatory disorders. J Cell Physiol 2018;233:98-106. [Crossref] [PubMed]
- Hinz B. Formation and function of the myofibroblast during tissue repair. J Invest Dermatol 2007;127:526-37. [Crossref] [PubMed]
- Li M, Yuan Y, Chen Q, et al. Expression of Wnt/β-Catenin Signaling Pathway and Its Regulatory Role in Type I Collagen with TGF-β1 in Scleral Fibroblasts from an Experimentally Induced Myopia Guinea Pig Model. J Ophthalmol 2016;2016:5126560.
- Ma M, Zhang Z, Du E, et al. Wnt signaling in form deprivation myopia of the mice retina. PLoS One 2014;9:e91086 [Crossref] [PubMed]
- Mo Y, Wang Y, Cao B, et al. Scleral TGF-β1 and Smad3 expression is altered by TCM Bu Jing Yi Shi Tablets in guinea pigs with form-deprivation myopia. J Tradit Chin Med Sci 2016;3:124-32.
- Chung Y, Fu E, Chin YT, et al. Role of Shh and TGF in cyclosporine-enhanced expression of collagen and alpha-SMA by gingival fibroblast. J Clin Periodontol 2015;42:29-36. [Crossref] [PubMed]
- Sánchez-Pozos K, Lee-Montiel F, Perez-Villalva R, et al. Polymerized type I collagen reduces chronic cyclosporine nephrotoxicity. Nephrol Dial Transplant 2010;25:2150-8. [Crossref] [PubMed]
- Gum SI, Kim YH, Jung JC, et al. Cyclosporine A inhibits TGF-beta2-induced myofibroblasts of primary cultured human pterygium fibroblasts. Biochem Biophys Res Commun 2017;482:1148-53. [Crossref] [PubMed]
- Hirota N, Ito T, Miyazaki S, et al. Gene expression profiling of lung myofibroblasts reveals the anti-fibrotic effects of cyclosporine. Tohoku J Exp Med 2014;233:283-93. [Crossref] [PubMed]
- Lin HJ, Wei CC, Chang CY, et al. Role of Chronic Inflammation in Myopia Progression: Clinical Evidence and Experimental Validation. EBioMedicine 2016;10:269-81. [Crossref] [PubMed]
- Fox LW. A practical treatise on ophthalmology. New York and London: D. Appleton and company, 1910.
- Shafer BM, Qiu M, Rapuano CJ, et al. Association between hay fever and high myopia in United States adolescents and adults. Eye Contact Lens 2017;43:186-91. [Crossref] [PubMed]
- Gao TT, Long Q, Yang X. Complement factors C1q, C3 and C5b-9 in the posterior sclera of guinea pigs with negative lens-defocused myopia. Int J Ophthalmol 2015;8:675-80. [PubMed]
- Herbort CP, Papadia M, Neri P. Myopia and inflammation. J Ophthalmic Vis Res 2011;6:270-83. [PubMed]
- Riddell N, Giummarra L, Hall NE, et al. Bidirectional expression of metabolic, structural, and immune pathways in early myopia and hyperopia. Front Neurosci 2016;10:390. [Crossref] [PubMed]
- Wang KK, Metlapally R, Wildsoet CF. Expression profile of the integrin receptor subunits in the guinea pig sclera. Curr Eye Res 2017;42:857-63. [Crossref] [PubMed]
- Tian XD, Cheng YX, Liu GB, et al. Expressions of type I collagen, alpha2 integrin and beta1 integrin in sclera of guinea pig with defocus myopia and inhibitory effects of bFGF on the formation of myopia. Int J Ophthalmol 2013;6:54-8. [PubMed]
- Riddell N, Crewther SG. Integrated comparison of GWAS, transcriptome, and proteomics studies highlights similarities in the biological basis of animal and human myopia. Invest Ophthalmol Vis Sci 2017;58:660-9. [Crossref] [PubMed]
- Wojciechowski R, Cheng CY. Involvement of multiple molecular pathways in the genetics of ocular refraction and myopia. Retina. 2018;38:91-101. [Crossref] [PubMed]
- Jiang B, Huo Y, Gu Y, et al. The role of microRNAs in myopia. Graefes Arch Clin Exp Ophthalmol 2017;255:7-13. [Crossref] [PubMed]
- Mei F, Wang J, Chen Z, et al. Potentially important microRNAs in form-deprivation myopia revealed by bioinformatics analysis of microRNA profiling. Ophthalmic Res 2017;57:186-93. [Crossref] [PubMed]
- Metlapally R, Park HN, Chakraborty R, et al. Genome-wide scleral micro- and messenger-RNA regulation during myopia development in the mouse. Invest Ophthalmol Vis Sci 2016;57:6089-97. [Crossref] [PubMed]
- Tkatchenko AV, Luo X, Tkatchenko TV, et al. Large-scale microRNA expression profiling identifies putative retinal miRNA-mRNA signaling pathways underlying form-deprivation myopia in mice. PLoS One 2016;11:e0162541 [Crossref] [PubMed]
- Wallman J, Winawer J. Homeostasis of eye growth and the question of myopia. Neuron 2004;43:447-68. [Crossref] [PubMed]
- Nickla DL, Wallman J. The multifunctional choroid. Prog Retin Eye Res 2010;29:144-68. [Crossref] [PubMed]
陈熹
中山大学中山眼科中心。眼科学博士,国际泪液与眼表协会会员、国际视觉发育视光师协会会员、百度认证深度学习工程师。担任《中华生物医学工程杂志》、《广州医科大学学报》等杂志青年编委,担任Neural Regen Res、《广东医学》等13个期刊审稿人。国内外会议发言近20次,以第一及共一作者在Cell Death Dis、Invest Ophthalmol Vis Sci等杂志上发表论文5篇。软件著作权4项,在申专利4项。(更新时间:2021/8/17)
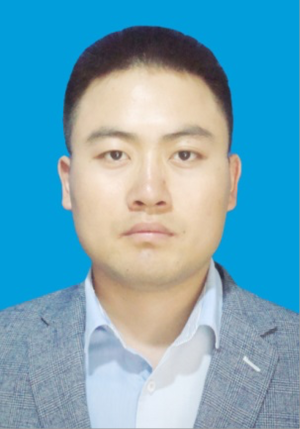
宋新志
甘肃省人民医院。眼科医学硕士,毕业于郑州大学,甘肃省人民医院眼科医生。主要从事白内障、青光眼及眼底病(眼底外科方向)的临床研究,近五年以第一作者发表中文核心期刊论文1篇,目前2篇SCI论文正在外审中。(更新时间:2021/8/12)
(本译文仅供学术交流,实际内容请以英文原文为准。)
Cite this article as: Backhouse S, Gentle A. Scleral remodelling in myopia and its manipulation: a review of recent advances in scleral strengthening and myopia control. Ann Eye Sci 2018;3:5.